The Biomarker Guide: Volume 1, Biomarkers and Isotopes in the Environment and Human History / Edition 2 available in Paperback, eBook
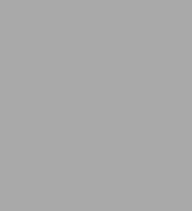
The Biomarker Guide: Volume 1, Biomarkers and Isotopes in the Environment and Human History / Edition 2
- ISBN-10:
- 0521786975
- ISBN-13:
- 9780521786973
- Pub. Date:
- 08/16/2007
- Publisher:
- Cambridge University Press
- ISBN-10:
- 0521786975
- ISBN-13:
- 9780521786973
- Pub. Date:
- 08/16/2007
- Publisher:
- Cambridge University Press
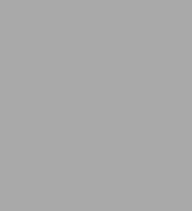
The Biomarker Guide: Volume 1, Biomarkers and Isotopes in the Environment and Human History / Edition 2
Buy New
$134.00Buy Used
$80.52-
-
SHIP THIS ITEM
Temporarily Out of Stock Online
Please check back later for updated availability.
-
Overview
Product Details
ISBN-13: | 9780521786973 |
---|---|
Publisher: | Cambridge University Press |
Publication date: | 08/16/2007 |
Edition description: | Revised Edition |
Pages: | 492 |
Product dimensions: | 7.40(w) x 9.65(h) x 0.98(d) |
About the Author
Senior Research Geochemist at ExxonMobil Research and Engineering Company.
A member of the Chevron Biomarker Group in the 1970s and 1980s during a time of pioneering research into the application of biomarker technology to petroleum exploration.
Read an Excerpt
Cambridge University Press
0521781582 - The Biomarker Guide - Second Edition I. Biomarkers and Isotopes in the Environment and Human History - by K. E. Peters, C. C. Walters and J. M. Moldowan
Excerpt
Part I
Biomarkers and isotopes in the environment
and human history
1 · Origin and preservation of organic matter
This chapter introduces biomarkers, the domains of life, primary productivity, and the carbon cycle on Earth. Morphological and biochemical differences among different life forms help to determine their environmental habitats and the character of the biomarkers that they contribute to sediments, source rocks, and petroleum. The discussion summarizes processes affecting the distribution, preservation, and alteration of biomarkers in sedimentary rocks. Various factors, such as type of organic matter input, redox potential during sedimentation, bioturbation, sediment grain size, and sedimentation rate, influence the quantity and quality of organic matter preserved in rocks. |
INTRODUCTION TO BIOMARKERS
Biological markers or biomarkers (Eglinton et al., 1964; Eglinton and Calvin, 1967) are molecular fossils, meaning that these compounds originated from formerly living organisms. Biomarkers are complex organic compounds composed of carbon, hydrogen, and other elements. They occur in sediments, rocks, and crude oils and show little or no change in structure from their parent organic molecules in living organisms. Sediments consist of unconsolidated mineral and organic detritus prior to lithification to form rock.
Biomarkers are useful because their complex structures reveal more information about their origins than other compounds. Unlike biomarkers, methane (CH4) and graphite (nearly pure carbon) are comparatively less informative because virtually any organic compound will generate these products when sufficiently heated. However, although methane and other hydrocarbon gases are simple compounds compared with biomarkers, they still contain useful information about their origin and geologic history, as discussed later in the text.
Three characteristics distinguish biomarkers from many other organic compounds:
- Biomarkers have structures composed of repeating subunits, indicating that their precursors were components in living organisms.
- Each parent biomarker is common in certain organisms. These organisms can be abundant and widespread.
- The principal identifying structural characteristics of the biomarkers are chemically stable during sedimentation and early burial.
Before proceeding with biomarkers, we need a general discussion of the different types of organism that contribute organic matter to the carbon cycle on Earth. This is followed by a discussion of the special circumstances required to preserve organic matter during and after sedimentation.
DOMAINS OF LIFE
The three major domains of life include the archaea and eubacteria (prokaryotes) (Woese et al., 1978) and the eukarya (eukaryotes or higher organisms) (Table 1.1). Unlike the prokaryotes, the eukaryotes contain a membrane-bound nucleus and complex organelles. For example, the organelles known as mitochondria and chloroplasts carry out important functions in energy generation and photosynthesis, respectively. Eukaryotic microorganisms include algae, protozoa, and fungi (molds and yeasts). All higher multicellular organisms are also eukaryotes.
The prokaryotes consist of millions of unicellular archaeal and eubacterial species, many not yet characterized. While eukaryotes are classified mainly by morphology, the prokaryotes are distinguished mainly by their diverse biochemistry and the habitats in which they grow (White, 1999). Their relatively simple shapes limit classification of prokaryotes based on morphology. Living prokaryotes should not be viewed as primitive. They are superbly adapted organisms that occupy all habitats. There is no universally accepted phylogeny for the prokaryotes.
Prokaryotes | Eukaryotes | ||
Eubacteria | Archaea | Eukaryota | |
Single-cell microorganisms | Most | All | Algae, protozoa |
Colonial with specialized cells | Cyanophyta, some others | None (?) | Algae, animals |
Multicellular with differentiated cells | None | None | Plants, animals |
Phototroph | Chemotroph | |
Autotroph | Photoautotroph | Chemoautotroph |
Heterotroph | Photoheterotroph | Chemoheterotroph |
The metabolism of prokaryotic species can be described as autotrophic, heterotrophic, phototrophic, chemotrophic, or by a compound name using multiple terms (Table 1.2) (Chapman and Gest, 1983). Autotrophs use carbon dioxide (CO2) as a sole source of carbon for growth and obtain their energy from light (photosynthetic autotrophs or photoautotrophs) or from the oxidation of inorganic compounds (chemosynthetic autotrophs or chemoautotrophs). In contrast, heterotrophs (photoheterotrophs and chemoheterotrophs) obtain both their carbon and their energy for growth from organic compounds. They are saprophytes, obtaining their nutrients from dead organic matter. Chemotrophic prokaryotes obtain their energy from the oxidation of inorganic compounds, such as H2, H2S, and NH3. Most chemotrophs are autotrophic, but some are heterotrophs (chemoheterotrophs), which use inorganic oxidation for energy but use organic matter for carbon as well as supplemental energy. Photosynthetic bacteria have the biochemistry for either anoxygenic photosynthesis (non O2-producing) or oxygenic photosynthesis (O2-producing). Most photosynthetic bacteria are autotrophs that fix CO2 (photoautotrophs), but some rely on organic matter for their carbon (photoheterotrophs). Adaptive prokaryotes switch their modes of metabolism depending on environmental conditions. Additional terms are commonly used to refine these basic descriptions. For example, methylotrophic bacteria are those heterotrophic organisms with the ability to utilize reduced carbon substrates with no carbon-carbon bonds (e.g. methane, methanol, methylated amines, and methylated sulfur species) as their sole source of carbon and energy. Methylotrophs that use methane are called methanotrophs. Acetogenic bacteria catalyze the reduction of two CO2 molecules to acetate in their energy metabolism. Diazotrophs have the ability to utilize N2 as a source of nitrogen for growth.
Oxygen tolerance, temperature, salinity, and pathology are commonly used to describe prokaryotes by habitat. Prokaryotes vary widely in their utilization and tolerance of oxygen. Obligate aerobes are prokaryotes that require oxygen. Facultative aerobes utilize oxygen, but can either tolerate its absence or switch to anaerobic metabolism, where oxygen is not required. Similarly, obligate anaerobes grow only in the absence of oxygen, while facultative anaerobes can tolerate oxygen. This tolerance usually depends on the presence of enzymes, such as superoxide dimutase and catalase, which can remove oxygen radicals. Many prokaryotes can survive wide ranges of temperature from below freezing up to boiling conditions. However, survival does not imply growth. Most prokaryotes have narrow temperature ranges for optimal growth. Prokaryotes have adapted to all temperatures at which water remains liquid. Prokaryotes that grow and thrive at very low temperatures (∼0 °C) are called psychrophiles. For example, mats dominated by benthic filamentous cyanobacteria grow immediately below permanent ice cover at Lake Hoare in the dry valley region of southern Victoria Land, Antarctica (Hawes and Schwarz, 1995).
Prokaryotes that flourish at moderate temperatures (0-45 °C) are called mesophiles. Thermophilic organisms have specially modified lipid membranes, proteins, and genetic material that allow them to survive at temperatures above 45 °C. Prokaryotes that grow at extremely low or high temperatures are called extreme psychrophiles or extreme thermophiles, respectively. Halophiles are organisms that tolerate high concentrations of dissolved salts. Extreme halophiles not only tolerate but also require high salt concentrations for growth. Descriptions of bacteria based on their pathogenicity and/or parasitism are important to the biomedical community.
The archaea (also called archaebacteria) are common in extreme environments and include extreme halophiles (hypersaline environments), thermophiles (hot), thermoacidophiles (hot and acidic), and methanogens (methane generated as a waste product of metabolism). Their diversity is now recognized in more moderate environments, such as soils and marine waters (Torsvik et al., 2002), as is their abundance in deep subsurface strata (e.g. Takai et al., 2001; Chapelle et al., 2002). Archaea are prokaryotes because they lack cell nuclei. While archaea share some common features with eubacteria and eukaryotes, they have genomes and biochemical pathways that are distinct from the other domains of life (Woese et al., 1978).
The morphologic differences between the three domains reflect fundamental differences in biochemistry (Table 1.3). Some of these differences control the types of biomarker that originate from these groups, as discussed later in this book. For example, steranes in petroleum originate from sterols in the cell membranes of eukaryotes. Prokaryotes use hopanoids rather than steroids in their cell membranes and these precursors account for the hopanes in petroleum.
Endosymbiotic theory suggests that the eukaryotes originated by an evolutionary fusion of different prokaryotes that originally lived together as symbionts (Schenk et al., 1997). According to this theory various organelles in eukaryotes, such as mitochondria, kinetosomes, hydrogenosomes, and plastids, originated as symbionts. For example, mitochondria and chloroplasts probably originated as aerobic non-photosynthetic bacteria and photosynthetic cyanobacteria, respectively. These organelles are morphologically similar to bacteria. Like bacteria, they are susceptible to antibiotics, such as streptomycin, they contain deoxyribonucleic acid (DNA) in the prokaryotic closed-circle form, and they have similar ribosomal ribonucleic acid (rRNA) phylogenetic sequences (Table 1.3).
Note: Viruses are non-cellular entities that lack the ability to reproduce without taking over the cellular apparatus of a host. For example, the T-4 bacteriophage consists of a protein coat and mechanical structure that injects the enclosed T-4 genetic material through the cell membrane of its bacterial host. Once inside, the T-4 genetic material reprograms the host cell to generate new T-4 virus. Viruses probably evolved from primitive life forms by loss of their original cellular components.
Endosymbiosis accounts for the presence of eubacterial genes within eukaryotic organisms. Some of these genes migrated to the nucleus of the host, while others remained within the organelle that was originally the symbiont (Palenik, 2002). Genomic comparisons of representatives from the three domains indicate that much of the prokaryotic DNA has been subject to horizontal gene transfer (HGT), the acquisition of new genes from other organisms typically via prokaryotic plasmids (Koonin et al., 2001). Plasmids are loops of DNA that are independent of the bacterial chromosome and can be exchanged between cells during conjugation. Genes acquired by horizontal transfer may range from being non-functional to being evolutionarily advantageous. For example, archaeal genes may have allowed some eubacteria to thrive under high-temperature conditions. Photosynthesis confers such an adaptive advantage that the genome of many photosynthetic bacteria appears to have resulted from massive HGT (Raymond et al., 2003). Woese (2002) advanced a theory that the three domains of life arose from early cellular evolution involving HGT from a communal pool.
Cellular domain | |||
Property | Eukarya | Bacteria | Archaea |
Cell configuration | Eukaryotic | Prokaryotic | Prokaryotic |
Nuclear membrane | Present | Absent | Absent |
Number of chromosomes | >1 | 1 | 1 |
Chromosome topology | Linear | Circular | Circular |
Murein in cell wall | No | Yes | No |
Cell-membrane lipids | Ester-linked glycerides; unbranched; polyunsaturated | Ester-linked glycerides; unbranched; saturated or monounsaturated | Ether-linked; branched; saturated |
Cell-membrane sterols | Yes | No1 | No2 |
Organelles (mitochondria and chloroplasts) | Yes | No3 | No |
Ribosome size | 80S (cytoplasmic) | 70S | 70S |
Cytoplasmic streaming | Yes | No | No |
Meiosis and mitosis | Yes | No | No |
Transcription and translation coupled | No | Yes | ? |
Amino acid initiating protein synthesis | Methionine | n-Formyl methionine | Methionine |
Protein synthesis inhibited by streptomycin and chloramphenicol | No | Yes | No |
Protein synthesis inhibited by diphtheria toxin | Yes | No | Yes |
Histones | Yes | No | Yes |
PRIMARY PRODUCTIVITY
Photosynthesis is the only significant means by which new organic matter is synthesized on Earth. It is the ultimate source for the biomass in almost all living organisms and accounts for most organic matter buried in sediments and rocks, including biomarkers. Many non-photosynthetic biosystems, such as methanotrophic communities (Michaelis et al., 2002), rely on recycling sedimentary carbon that originally was fixed by photosynthetic organisms. In some environments, organic matter originates from non-photosynthetic chemoautotrophy (e.g. deep-sea hydrothermal vents), but their contribution to Earth's pool of sedimentary carbon is minor.
Bacterial and green-plant photosynthesis can be represented by the following generalized reaction:
Display matter not available in HTML version
The formula CH2O stands for organic matter in the form of a carbohydrate, such as the sugar glucose (C6H12O6). Polysaccharides (i.e. polymerized sugars) are the main form in which photosynthesized organic matter is stored in living cells. Respiration is the reverse of Reaction (1.1), where energy for cell function is released by oxidizing polysaccharides.
Because energy from light is required for photosynthesis, photosynthetic organisms are restricted to the land and the photic zone in lakes and the ocean. Light intensity diminishes rapidly with water depth in the oceans and lakes, limiting the depths of the photic zone to less than ∼100 m. Phototrophs are organisms that derive energy for photosynthesis from light (Table 1.2). Phototrophic microorganisms are the most important photosynthesizers in aquatic environments, while higher plants dominate the land. This fundamental difference affects the types of organic matter deposited in sediments from these environments. Chemosynthesis, where chemical energy is released by oxidation of reduced substrates, can occur in deep water beyond the photic zone.
Figure 1.1. Generalized redox cycle for organic carbon. Production of new organic matter by photosynthetic fixation of carbon from CO2 (primary productivity) can occur with (aerobic, left) or without (anaerobic, right) oxygen as a by-product. Respiration and other processes result in the nearly complete oxidation of this organic matter back to CO2. Reprinted with permission by ChevronTexaco Exploration and Production Technology Company, a division of Chevron USA Inc.
Image not available in HTML version
When organisms grow phototrophically, the rate of fixation of carbon from CO2 into carbohydrate exceeds the rate of respiration. The whole cycle on Earth (Figure 1.1) is based on a net positive balance in the rate of photosynthesis over the rate of respiration.
Green-plant photosynthesis is a variation of Reaction (1.1):
Display matter not available in HTML version
This process is an oxidation-reduction (redox) reaction. The oxidation in Reaction (1.2) is:
Display matter not available in HTML version
Water is split to produce reducing power (hydrogen atoms), with O2 as a by-product. The reduction reaction is:
Display matter not available in HTML version
As shown above, the H2O product originates by reduction of CO2. The necessary hydrogen atoms originate from a donor molecule or reductant (in this case, H2O) and are transferred to an acceptor molecule or oxidant, CO2. After the reaction, the donor molecule is referred to as oxidized, while the acceptor is reduced.
In green plants and cyanobacteria (Reaction 1.2), H2A is H2O and 2A is O2. In bacteria, H2A is some oxidizable substrate and 2A is the product of its oxidation. For example, in sulfur bacteria H2A is hydrogen sulfide (H2S) and A is sulfur:
Display matter not available in HTML version
Note: The equation for bacterial photosynthesis clearly shows it to be an oxidation-reduction reaction. In bacteria, water is one of the products of the reduction of CO2 and it differs from the oxidizable component, H2S. In green plants, the oxidant and the product of the reduction cannot be readily distinguished without isotopic labeling experiments because both consist of water (reaction 1.2).
In addition to light, the main limitation on planktonic primary productivity is the availability of nutrients, particularly nitrates and phosphates. Nitrogen fixation, or the conversion of atmospheric N2 to NO3-, occurs mainly due to soil microbes, while phosphate originates from weathering of continental rocks. Thus, both nitrate and phosphate in lakes and the oceans depend on run-off from land. Because they are surrounded by land, lakes commonly contain more nutrients than most ocean areas and therefore have higher primary productivity (Table 1.4). Open-ocean areas far from land constitute ∼90% of the ocean surface but have few nutrients and very low productivity (Table 1.4). Vertical recycling of nutrients is negligible because of thermal and salinity stratification, which separate the shallow- and deep-water masses. In more productive coastal and upwelling areas of the oceans, these nutrients are rapidly sequestered by phytoplankton in the photic zone and rise through the various trophic levels in the food chain. The combined photosynthetic process to convert inorganic carbon and these nutrients to biomass and oxygen in diatoms is as follows (Redfield, 1942):
Display matter not available in HTML version
Upwelling occurs mainly in shallow (<200 m) coastal waters and is the principal mechanism where deeper, nutrient-rich waters are recycled into the photic zone. Upwelling areas constitute ~0.1% of the ocean area, but have elevated productivities due to a combination of nutrient supply from land run-off and efficient vertical recycling of nutrients (Table 1.4). For example, upwelling and recycling of nitrates and phosphates in offshore Peru is related to wind stress. Prevailing winds push surface water northward at the same time that the Coriolis force deflects it to the west, resulting in overturn and upwelling of cold, nutrient-rich subsurface water. Many Phanerozoic phosphorites were deposited in low-latitude upwelling zones on continental shelves, such as the Permian Phosphoria Formation (e.g. Claypool et al., 1978; Maughan, 1993).
Annual productivity | |
Aquatic ecosystem | (g carbon/m2/year) |
Open ocean | 25-50 |
Coastal ocean | 70-120 |
Upwellings | 250-350 |
Oligotrophic lake | 4-180 |
Mesotrophic lake | 100-310 |
Eutrophic lake | 370-640 |
Chlorophyll plays a critical role in converting the energy of light to chemical energy stored in the products of photosynthesis. This stored energy is released when the products are brought together and undergo combustion or respiration. Forest fires and warm muscles caused by exertion both utilize this type of stored energy.
All photosynthetic organisms have some type of chlorophyll. Chlorophyll a (e.g. see Figure 3.23) is the principal chlorophyll in higher plants, most algae, and cyanobacteria. Other chlorophylls absorb light in different ranges of wavelength. For example, purple and green bacteria contain bacteriochlorophylls. The various chlorophylls differ mainly in the alkyl groups attached to the tetrapyrrole ring. Chlorophylls tend to absorb light most efficiently in the red portion of the visible spectrum. Because red wavelengths do not penetrate deeply into water, aquatic plants commonly use different carotenoids as accessory pigments to increase the range of wavelengths that can be used for photosynthesis. Accessory pigments, such as carotenoids and xanthophylls, are involved at least indirectly with the capture of light and occur with chlorophylls in many phototrophic organisms. Biomarkers derived from chlorophylls, bacteriochlorophylls, and accessory pigments in living autotrophs occur in ancient rocks and petroleum and can be used to help identify past sources of organic matter.
SECONDARY PRODUCTIVITY
Much of the primary organic matter created by photosynthetic or chemosynthetic autotrophs undergoes degradation by other organisms during the secondary production of organic matter. Heterotrophic microbes in the water column, sediments, and rock continually degrade and rework primary aquatic and terrigenous organic matter. Heterotrophic microbes reduce the mass of primary organic matter preserved in sediments, while contributing some of their own characteristic secondary organic matter. For example, methane can be produced from primary organic matter in surficial anoxic sediments by methanogenic bacteria (Figure 1.1). However, these methanogens also create various characteristic compounds, such as 2,6,10,15,19-pentamethylicosane and squalane, which remain in the sediments (e.g. see Figure 2.15). Carbon dioxide is a product of various organisms that utilize fermentation, anaerobic respiration, or aerobic respiration to produce energy. As another example, when methane moves into oxic environments, methylotrophic bacteria can oxidize it to CO2 (Figure 1.1). Details on these various organisms can be found in any modern discussion of microbial metabolism (e.g. Brock and Madigan, 1991).
Fossil fuels represent organic matter that has been temporarily removed from the carbon cycle due to burial in the lithosphere. Sedimentation and early burial normally result in the complete destruction of primary organic matter by heterotrophic degradation and other processes, such as chemical oxidation. One can view all fossil fuels, including finely disseminated organic matter, coal, and petroleum, as excess organic carbon that escaped an otherwise remarkably efficient carbon cycle due to burial (Figure 1.1). This excess organic carbon represents a small leak in the carbon cycle. It is estimated that during Earth history, 0.1% of the carbon produced as biomass by plants (primary productivity) becomes preserved in sediments and available for petroleum-related processes (Tissot and Welte, 1984). About 0.6% of the organic carbon produced in marine basins is preserved (Hunt, 1996, p. 113). Metamorphism, uplift, erosion, and combustion of fossil fuels eventually return stored organic carbon to the cycle. Organic matter, including biomarkers, is best preserved in sediments under special circumstances, as described below.
Figure 1.2. Generalized evolution of organic matter during and after sedimentation. Many biomarkers survive diagenesis and much of catagenesis prior to their complete destruction during late catagenesis and metagenesis (left). Depth scale can vary depending on different factors, including geothermal gradient and type of organic matter. High primary productivity by plants, anoxia (lack of oxygen), and other factors favor preservation of organic matter during diagenesis (right). Potential source rocks can become effective source rocks during catagenesis. Reprinted with permission by ChevronTexaco Exploration and Production Technology Company, a division of Chevron USA Inc.
Image not available in HTML version
PRESERVATION OF ORGANIC MATTER
Biomarkers exist because their basic structures remain intact during sedimentation and diagenesis (Figure 1.2). In this book, the term "diagenesis" refers to the biological, physical, and chemical alteration of organic matter in sediments prior to significant changes caused by heat (typically <50 °C).
Catagenesis is the process by which the organic matter in rocks is thermally altered by burial and heating at temperatures in the range of ∼50-150 °C under typical burial conditions requiring millions of years. During catagenesis, biomarkers undergo structural changes that can be used to gauge the extent of heating of their source rocks or oils expelled from these rocks. Furthermore, because biomarkers represent a distinctive set of contributing organisms, their distribution in an effective source rock is a fingerprint that can be used to relate the rock to expelled crude oil that may have migrated many kilometers. At temperatures in the range of ∼150-200 °C, prior to greenschist metamorphism, organic molecules are cracked to gas in the process called metagenesis. Biomarkers are severely diminished in concentration or completely destroyed because of their instability under these conditions. Deep hydrocarbon gas accumulations can result from (1) cracking of highly mature particulate organic matter, (2) decomposition of residual oil in petroleum source rocks, and (3) secondary cracking of oil in reservoir rocks (Lorant and Behar, 2002). No convincing evidence has been found for deep, commercial methane accumulations that originated by inorganic processes, such as mantle outgassing (see Chapter 9).
Biomarkers are powerful geochemical tools because many are highly resistant to biodegradation. For example, biodegraded seep oils and asphalts commonly contain unaltered biomarkers that can be used for comparisons with non-biodegraded oils.
ORGANIC COMPONENTS IN ROCKS
In sedimentary rocks that have undergone diagenesis, the organic matter consists of kerogen, bitumen, and minor amounts of hydrocarbon gases. Kerogen is non-hydrolyzable particulate organic debris that is insoluble in organic solvents and consists of mixtures of macerals and reconstituted degradation products of organic matter (Durand, 1980). Macerals are recognizable remains of different types of organic matter that can be differentiated under the microscope by their morphologies (Stach et al., 1982; Taylor et al., 1998). They are analogous to minerals in a rock matrix, but they differ in having less well-defined chemical compositions. Part of the kerogen represents reconstituted low-molecular-weight degradation products of biomass (e.g. Durand, 1980; Tissot and Welte, 1984). In addition, Tegelaar et al. (1989) list recognized biomacromolecules (e.g. cellulose, proteins, and tannins) and rank their relative potential for preservation by incorporation into kerogen through cross-linkage during diagenesis. Transmission electron microscopy reveals that many kerogens described as amorphous using standard microscopy actually consist of bundles of thin lamellae (∼10-30 nm) called ultralaminae, which represent insoluble biomacromolecules inherited from the outer cell walls of microalgae (Largeau et al., 1990; Derenne et al., 1993). Kerogen macerals generally reflect local (autochthonous) primary and secondary production of organic matter. However, many sediments contain appreciable transported (allochthonous) organic matter, such as land-plant detritus or pollen, soot from fires, or soil carried by rivers or wind into marine or lacustrine settings (Gogou et al., 1996).
Bitumen consists of in situ hydrocarbons and other organic compounds that can be extracted from fine-grained sedimentary rocks using organic solvents. Biomarkers occur both free in the bitumen and chemically bound to the kerogen in petroleum source rocks. They also occur in crude oils that migrate from the fine-grained source rocks to reservoir rocks. Petroleum is a general term that refers to solid, liquid, and gaseous materials composed dominantly of chemical compounds of carbon and hydrogen, including bitumen, hydrocarbon gases, and crude oil. Petroleum accounts for ∼63% of the total worldwide energy consumption (US Geological Survey, 2000).
The quantity and quality of organic matter preserved during diagenesis of sediment ultimately determine the petroleum-generative potential of the rock. Various factors play a role in the preservation of organic matter during sedimentation and burial, notably organic productivity, oxygen content of the water column and sediments, water circulation, sediment particle size, and sedimentation rate (Demaison and Moore, 1980; Emerson, 1985; Meyers, 1997). For example, anoxia, sediment size, primary productivity, organic source material, and contemporaneous diagenesis in the water column all contribute to organic matter accumulation and preservation in organic-rich shales from the Upper Devonian to Lower Carboniferous Exshaw Formation of southern Alberta (Caplan and Bustin, 1996). The relative importance of these factors remains controversial and probably differs between depositional environments.
OXIC VERSUS ANOXIC DEPOSITION
Aerobic microbes rapidly oxidize organic matter from dead plants or animals under atmospheric conditions. Aqueous sedimentation of organic matter can occur under various redox conditions, controlled mainly by the availability of molecular oxygen. The different redox conditions and their corresponding microbial biofacies (determined by metabolism) can be described using the terms in Table 1.5.
Oxygen content (ml/l) | |||
Rhodes and | Tyson and | ||
Depositional | Microbial | Morse | Pearson |
environment | biofacies | (1971) | (1991) |
Oxic | Aerobic | >1 | 2-8 |
Dysoxic | Dysaerobic | 0.1-1 | 0.2-2 |
Suboxic | Quasi- | 0.1-1 | 0-0.2 |
anaerobic | |||
Anoxic | Anaerobic | 0 | 0 |
Under an oxic water column, aerobic bacteria and other organisms degrade organic matter settling from the photic zone (Figure 1.4, left). Normal seawater contains ∼6-8 ml oxygen/liter. Respiratory processes create biological oxygen demand (BOD). If sufficient organic matter remains after exhausting all available oxygen, then anaerobic organisms continue to oxidize the organic matter by using other oxidants, such as nitrate or sulfate. The boundary between aerobic and anaerobic metabolism (oxic versus anoxic environments) can occur in the water column or in the bottom sediments. Benthic sediments that contain interstitial oxygen are commonly bioturbated by metazoa, including multicellular burrowing organisms, such as clams and worms. Oxic sediments that are preserved in the geologic record have massive textures without lamination.
Lakes and oceanic basins can become depleted in oxygen (stagnant) due to the combined effects of BOD and limited recharge by oxygenated water. Water recharge is controlled by various factors, including basin geometry, water temperature, and salinity gradients. For example, a thermocline is a layer of water where the temperature decrease with depth is greater than that in the underlying and overlying water (Figure 1.3). Thermoclines can develop in lakes and the ocean when the air temperature is high and the shallow water receives more heat than it loses by radiation and convection. The surface water warms and a shallow negative temperature gradient develops. Mixing of the surface waters by wind may lower the surface temperature, but the net effect is downward transport of heat and formation of an isothermal layer that is warmer than the underlying water. A strong thermocline thus develops between the isothermal surface layer and cooler water below. In the oceans, warm surface waters generally extend to 150-300-m depth. The underlying thermocline varies from 300 to 900 m thick. Temperature decreases more slowly below the thermocline and generally reaches 1-4 °C near the ocean bottom.
© Cambridge University Press